Light
Lichtschein is a redirect to this article. For the Austrian hockey and ice hockey goalie, see Fritz Lichtschein.
The title of this article is ambiguous. For other meanings, see Light (disambiguation).
Light is a form of electromagnetic radiation. In the narrower sense, only the parts of the entire electromagnetic spectrum visible to the human eye are meant. In a broader sense, electromagnetic waves of shorter wavelength (ultraviolet) and longer wavelength (infrared) are also included.
The physical properties of light are described by different models: In ray optics, the straight-line propagation of light is illustrated by "light rays"; in wave optics, the wave nature of light is emphasized, which can also explain diffraction and interference phenomena. Finally, in quantum physics, light is described as a stream of quantum objects called photons (illustratively also called "light particles"). Quantum electrodynamics provides a complete description of light. In vacuum, light propagates at the constant speed of light of 299,792,458 m/s. When light encounters matter, it can be scattered, reflected, refracted and slowed down or absorbed.
Light is the sensory stimulus adequate for the human eye. The intensity of the light is perceived as brightness, the spectral composition as color.

Sunlight coming through the slats of a barn
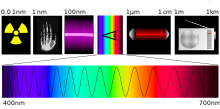

Visible light is part of the electromagnetic spectrum. (From short to long wavelengths: Gamma rays, X-rays, UV, visible light, IR, microwaves, radio waves. The wavelengths given are only approximate).
History
Until well into modern times, it was largely unclear what light actually was. It was partly believed that brightness filled space without any time delay. Pythagoras believed that "hot rays of vision" emanate from the eyes and are pushed back by other objects. If this were true, man should be able to see even in the dark. However, there have also been ideas since ancient times that light is emitted from the light source at a finite speed.
Galileo Galilei was one of the first to try to seriously measure the speed of propagation of light, but without success. The means at his disposal were far too crude for this. It was only Ole Rømer who succeeded in doing so, using observation data from the moons of Jupiter in 1675. It is true that the deviation of his measured value from the actual value (approx. 2.3 - 108 m/s) was around 30 %. However, Rømer's real achievement was to prove that light propagates at a finite speed. Rømer's measurement was made more and more precise in the course of the following 200 years by increasingly sophisticated methods (especially by Hippolyte Fizeau and Léon Foucault). The nature of light, however, remained unexplained. In the 17th century, Isaac Newton's corpuscle theory attempted to explain the propagation of light by the motion of small particles. This made it possible to understand reflection, but not some other optical phenomena, such as diffraction, which is clearly a wave phenomenon. At the same time, Christiaan Huygens and others established the wave theory of light, but it was not until the beginning of the 19th century, after the double-slit experiments of Thomas Young, that it became increasingly accepted.
In 1846, Michael Faraday was the first to prove that light and magnetism are two interrelated physical phenomena. He published the magneto-optical effect he had found, now known as the Faraday effect, under the title On the Magnetization of Light and the Exposure of Magnetic Force Lines.
In 1864, James Clerk Maxwell formulated the basic equations of electrodynamics, which are still valid today, and realized that this predicted the existence of free electromagnetic waves. Since their predicted speed of propagation matched the known speed of light, he concluded that light was probably an electromagnetic wave. He assumed (as did almost all physicists at the time) that this wave could not exist in empty space, but needed a propagation medium. This medium, which would have to fill the entire universe, was called the aether.
With the electromagnetic theory of light based on it, almost all questions about light seemed to be clarified in the late 19th century. However, on the one hand the postulated ether could not be proven (see Michelson-Morley experiment), which finally opened the door to the special theory of relativity. On the other hand, the photoelectric effect, among other things, seemed to contradict the wave nature of light. Thus a radically new view of light emerged, founded by the quantum hypothesis of Max Planck and Albert Einstein. The core of this hypothesis is wave-particle duality, which now describes light no longer exclusively as a wave or exclusively as a particle, but as a quantum object. As such, it combines properties of wave and of particle without being one or the other and thus eludes our concrete view. This gave rise to quantum physics at the beginning of the 20th century and later to quantum electrodynamics, which still represents our understanding of the nature of light today.
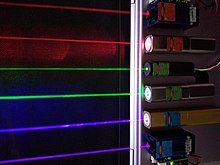

Different colored lasers
Science
Physics
In the following, the most important models for the description of light are presented. Like all models in physics, the ones listed here are also limited in their scope. A complete description of the phenomenon "light" according to our present knowledge can only be provided by quantum electrodynamics.
Light as an electromagnetic wave
→ Main article: Electromagnetic wave
In classical electrodynamics, light is understood as a high-frequency electromagnetic wave. In the narrower sense, "light" is only the part of the electromagnetic spectrum visible to the human eye, i.e. wavelengths between approx. 380 and 780 nm. This corresponds to frequencies of about 385 to 790 THz. It is a transverse wave, where the amplitude is given by the vector of the electric field or the magnetic field. The direction of propagation is perpendicular to it. The direction of the field vector or
field vector is called the direction of polarization. In unpolarized light, the radiation field is composed of waves of all polarization directions. Like all electromagnetic waves, visible light propagates in a vacuum at the speed of light .
The wave equation of this electromagnetic wave can be derived from Maxwell's equations. From this, there is a simple relationship between the speed of light, the magnetic field constant μ and the electric field constant ε
:
in a vacuum,
in the medium.
Obviously, the speed of light - more precisely: the phase velocity of light - in media depends on their material properties. These can be summarized in the refractive index . In general, it is frequency-dependent, which is called dispersion. Among other things, the ability of a prism to separate light into its spectral components is based on this. Short-wave blue light (< 450 nm) is refracted more strongly than long-wave red light (> 600 nm) under normal dispersion.
Ray Optics
→ Main article: Geometric optics
Ray optics (also geometrical optics) makes use of the approximation that the propagation of light can be illustrated by straight "rays". This approximation is especially justified when the dimensions of the experimental setup are large compared to the wavelength of the light. Then all diffraction phenomena can be neglected. The link between wave optics and ray optics is the wave vector whose direction coincides with the direction of the light beam. Ray optics is particularly well suited to describe phenomena such as light and shadow, reflection or refraction. Therefore, it can be used to explain the function of many optical devices (pinhole camera, magnifying glass, telescope, microscope). In particular, the imaging laws are also the basis for understanding the refractive apparatus in the human eye.
Principles of rays
- Light rays always propagate in a straight line and change their direction only when they hit a body (by reflection, refraction or scattering), disregarding the deflection of light by heavy masses observed in astronomy (gravitational lensing effect).
- Light rays can pass through each other without influencing each other.
- The path of light is reversible. This means that any ray path would satisfy all optical laws even if the direction of propagation of light were reversed.
Reflection
Light is reflected from reflective surfaces (bare metal, water surface) according to the law of reflection. The incident and the outgoing beam as well as the perpendicular on the reflecting surface lie in one plane. The angle of incidence and the angle of reflection are equal. The ratio of the reflected light intensity to the incident light intensity is called the reflectance and depends on the material and wavelength. The reflectance indicates what percentage of the luminous flux falling on a surface is reflected.
Refraction
Light is refracted at the interface between two media of different optical density, i.e. a beam changes its direction at this interface. (For the sake of completeness, it should be said that reflection always occurs to a greater or lesser extent at such an interface). The law of refraction of Snellius states:
The incident and the refracted beam as well as the perpendicular on the interface lie in one plane. The angle between the perpendicular and the light beam is smaller in the medium that has the higher refractive index.
The exact angles δ can be calculated by the refractive indices
the media involved:
.
If the incident beam from the optically denser medium hits the interface at a flat angle, there is no real angle for the refracted beam that satisfies this condition. In this case, total internal reflection occurs instead of refraction.
Wave optics
→ Main article: Wave optics
Wave optics is based on the principle of Huygens and Fresnel.
Each point of a wave front is the starting point of an elementary wave. A wave front results from the superposition of these elementary waves.
Elementary wave in this context means a spherical wave emanating from a certain point. Wave fronts are the surfaces of the same phase. The distance between two successive wavefronts is thus the wavelength. The wavefronts of a plane wave are thus planes, while the wavefronts of elementary waves are concentric spherical surfaces. The direction of propagation (i.e. the direction of the wave vector) always forms a normal to the wave front. Wave optics can be used to understand all phenomena of diffraction and interference. It is also suitable for deriving the laws of reflection and refraction. Wave optics does not contradict ray optics, but extends and deepens it.
Historically, the wave optics of Huygens and Fresnel already anticipates an essential insight of electrodynamics: light waves are electromagnetic waves.
Photons
→ Main article: Photon
In quantum physics, light is no longer regarded as a classical wave, but as a quantum object. According to this, light is composed of individual discrete energy quanta, the so-called photons. A photon is an elementary particle, more precisely an elementary boson with a mass of 0, which always travels at the speed of light .
It carries an energy of
Where ν is the frequency of light and
is Planck's quantum of action with
.
The photon has a momentum of
where λ is the wavelength of the light.
A photon is either absorbed and emitted as a whole or not at all. So it is "countable" like a particle. Nevertheless, everything that has been said here so far about the wave properties of light remains valid. This strange behavior of photons, but which all other quantum objects also exhibit, has been labeled "wave-particle duality": Quantum objects are neither like classical particles nor like classical waves. Depending on the point of view, they show properties of one or the other.
In today's most common interpretation of quantum mechanics (Copenhagen interpretation), one cannot predict the exact location of a photon a priori. One can only make statements about the probability with which a photon will hit a certain place. This probability density is given by the absolute square of the amplitude of the light wave.
Historically, the quantum mechanical description of light became necessary because some phenomena could not be explained by purely classical electrodynamics:
- If one imagines a thermal light source (ideally a black body) as a collection of many atomic oscillators in equilibrium with the radiation field, a classical derivation would lead to the "UV catastrophe", short-wave radiation would have to be much more strongly represented in the spectrum of the black body than it is. (Rayleigh-Jeans law)
- Classical electrodynamics would predict that the energy of electrons released in the photoelectric effect is proportional to the intensity of the absorbed radiation. In fact, however, it is proportional (except for a constant summand) to the frequency of the radiation. This relationship cannot be understood classically.
- Sensitive detectors (e.g. photomultipliers) do not receive a constant, uniformly low intensity at weak irradiation, but individual signals that are very narrowly limited both spatially and temporally.
- The spectrum of X-ray bremsstrahlung has a short-wavelength limit that is directly related to the energy of the electrons used to produce it.
Interaction with matter
·
Reflection
·
Scatter
·
Absorption
·
Refraction
·
Birefringence
·
Optical activity
·
Photoelectric effect
In addition to the phenomena described earlier in this article.
- Refraction
- Reflection
there are numerous other interactions between light and matter.
- Absorption: The energy of the incident light is swallowed by a body. This can cause an electron to be lifted to a higher energy level, the body to heat up, etc. When radiation is absorbed regardless of its wavelength, the body appears black. If only a part of the spectrum is absorbed, the remaining parts of the spectrum determine the color of the body (subtractive color mixing). In the case of electronic excitation, the energy can also be emitted again in the form of radiation. This is referred to as spontaneous emission, fluorescence or - if the process is significantly delayed in time - phosphorescence.
- Birefringence: Some materials split a beam of light into two beams of different polarization.
- Optical activity: Certain media can rotate the plane of polarization of polarized light.
- Photoelectric effect: The photons release electrons from the irradiated body.
- Scattering: The light changes its propagation, but not as in the case of reflection in a defined direction, but diffusely in all possible spatial directions. Depending on the scattering body, a distinction is made between Compton scattering (by free electrons), Rayleigh scattering (by bound electrons without energy transfer), Raman scattering (by bound electrons with energy transfer), Mie scattering (by particles whose expansion is in the order of magnitude of the wavelength).
Light sources
In principle, a distinction is made between thermal and non-thermal radiators. The former obtain the energy for radiation emission from the thermal movement of their particles. Examples are candle flames, glowing bodies (filament of a light bulb) and the sun. The spectrum of a thermal radiator is continuous, i.e. all wavelengths occur. According to Planck's radiation law, the spectral components depend exclusively on the temperature, but, apart from the spectral emissivity, not on the material of the radiator.
In contrast, non-thermal light sources do not have a continuous spectrum, but a line or band spectrum. This means that only very specific wavelengths are emitted. Line spectra occur in gas discharge tubes, band spectra in light-emitting diodes, auroras or fireflies. The energy sources for the radiation here are electric current, particle radiation or chemical reactions. Line spectra are often characteristic for certain substances.
The laser occupies a special position among light sources. Laser light is almost monochromatic (it consists almost only of one wavelength), more or less coherent (there is a fixed phase relationship between several wave trains) and often polarized.
Cherenkov radiation is produced by the movement of charged particles through a transparent dielectric when the particle velocity is higher than the speed of light in the dielectric. It is the analogue of sonic booms and can be observed, for example, in swimming pool reactors and nuclear power plant decay pools.
Light receiver
- The intact sense of sight is the simplest evidence. Accordingly, the eye plays an important role in the direct observation of processes involving light.
- Photographic film plays a major role in the study of the nature of light: one can document the lowest light intensities of distant stars and their spectra through long exposures. Photographic layers can be sensitized to different parts of the spectrum. In the meantime, however, photographic film is being replaced more and more by image sensors.
- Optical radiation detectors mostly use the external (photocell, vidicon, image intensifier, photomultiplier) and internal (semiconductor detectors such as photodiode, phototransistor, photoresistor) photoelectric effect. Complex sensors (line sensors and image sensors), which also serve as recording elements in scanners and digital cameras, also work with semiconductor detectors. Color sensors work with several photodetectors located behind different filters.
- Fluorescence can be used to detect ultraviolet and also infrared (after two-photon absorption) by evaluating the resulting visible light.
- Light can also be detected by its thermal effect. The bolometers used in astronomy to measure the radiant power of astronomical light sources, as well as thermal power meters for high-power laser beams, are based on this principle.
Biology
Light as an eco-factor
Light represents the most important eco-factor for plants - apart from the availability of water - because it provides energy for photosynthesis. The light energy absorbed by the chlorophyll molecules in the chloroplasts is used to split water molecules (photolysis) and thus produce reducing agents for photosynthesis. These are used in a second step to gradually reduce carbon dioxide eventually to glucose, from which starch, among other things, is built. The oxygen produced during photolysis is released into the atmosphere as a residual substance. The sum reaction equation of photosynthesis is:
The formation of organic compounds from carbon dioxide is called carbon dioxide assimilation. Organisms that are able to do this with the help of light are called photo-autotrophs. In addition to vascular plants, mosses, algae and some bacteria, such as cyanobacteria and purple bacteria, also belong to this group. All heterotrophic organisms are dependent on this assimilation, because they can only cover their energy requirements from organic compounds that they have to take up with food.
The competition of plants for light is noticeable in the "cane structure" of the forest and the associated specialisation of light and shade plants or in the seasonal succession of different aspects. In water bodies, only the light-flooded uppermost layer, the nutrient layer, serves the formation of biomass and oxygen, mainly by phytoplankton. Because many animals and protozoa find good living conditions here due to the high food supply and the comparatively high oxygen content of the water, they are attracted by the light.
The sense of light or sight is one of the most important senses for many animals. It is used for orientation in space, to control the day-night rhythm, to detect danger, to track down prey and to communicate with conspecifics. Therefore, in the course of evolution, a wide variety of light-sensing organs have developed in the most diverse taxa. These range from the simple eye spots of Euglena to simple pigment fields to complex compound eyes and lens eyes. Only a few animals are completely insensitive to light stimuli. This is at most the case when they live in complete darkness, like cave animals.
For both predators and prey, it is advantageous not to be seen. Adaptations to this are camouflage and night activity. Surprisingly, however, many creatures have themselves developed the ability to glow. The best known example is the firefly. However, this phenomenon of bioluminescence can also be found in deep-sea fish, luminescent crabs, fungi (e.g. Hallimasch) or bacteria. The benefits of bioluminescence are mainly explained by intra-species communication, deterring predators and attracting prey.
Light as a sensory stimulus
The light that falls into the human eye is projected through the refractive apparatus (consisting of cornea, anterior and posterior eye chamber, lens and vitreous body) onto the retina. There, a real, upside-down image is created (comparable to the process in a photo camera). This stimulates the photoreceptors (= light-sensing cells) located in the retina, which convert the stimulus into an electrical signal. This signal is transmitted to the brain via the optic nerve, into which the individual nerve strands of the retina flow. There, the upside-down images of our environment are then "straightened out" in real time.
Light intensity is perceived as brightness. The eye can adapt to the intensities - which are many powers of ten - by means of various mechanisms (see adaptation). The perceived brightness is related to the actual intensity via the Weber-Fechner law.
The spectral composition of the light stimulus is perceived as color, and the human eye can detect light with wavelengths between approximately 380 nm and 750 nm. If white light is separated (through a prism), the wavelengths appear as the colours of the rainbow.
Wavelength ranges of the spectral colors | |||||
(about) hue | Wavelength λ | Wave frequency ν | Energy E per photon in eV | Wavenumber ν | |
Purple | 380–420 | 789,5–714,5 | 3,26–2,955 | 26.316–23.810 | |
Blue | 420–490 | 714,5–612,5 | 2,95–2,535 | 23.810–20.408 | |
Green | 490–575 | 612,5–522,5 | 2,53–2,165 | 20.408–17.391 | |
Yellow | 575–585 | 522,5–513,5 | 2,16–2,125 | 17.391–17.094 | |
Orange | 585–650 | 513,5–462,5 | 2,12–1,915 | 17.094–15.385 | |
Red | 650–750 | 462,5–400,5 | 1,91–1,655 | 15.385–13.333 |
It should be noted that this table only applies to monochromatic light. Mixed colors may produce different color impressions. For example, a mixed color of monochromatic green and red light appears yellow to the human sense of sight, while a mixture of monochromatic red and blue light appears as magenta. The color impression magenta, unlike yellow, cannot be caused by any monochromatic light. The color brown, on the other hand, which is generally thought to be a mixed color, can be produced by monochromatic orange if its intensity is weak compared to its surroundings.
The retina of the eye is equipped with various sensory cells: The rods have a broad spectral responsiveness and are characterized by a high sensitivity. They are therefore specialized in twilight vision, but cannot distinguish colors. The cones, on the other hand, which are adapted to stronger intensities, occur in three different types, each of which has its response optimum at a different wavelength. Their interconnection ultimately makes color vision possible.
In both rods and cones, the visual process is based on the absorption of photons by the visual pigment (in the case of rods: rhodopsin). The ligand retinal undergoes isomerization, which causes rhodopsin to decay and initiates the signaling cascade of phototransduction. The resulting hyperpolarization of the cell membrane of the rods and cones causes an electrical signal that is passed on to the downstream neurons.
See also: visual pathway
In addition to cones and rods, there is a third light receptor, the melanopsin-containing ganglion cells. These receptors are particularly sensitive to blue light and are involved in the control of the internal clock. Their discovery at the turn of the millennium forced the development of lighting concepts for interiors similar to daylight, such as Human Centric Lighting.
The performance of the light-sensing organs of other creatures differs in part considerably from that of humans. Most mammals have rather underdeveloped color vision. Birds, on the other hand, have more cone types and can accordingly distinguish more colours than humans. Bees, while more or less insensitive to long-wave (red) light, can perceive very short-wave UV light, which is invisible to humans. They can also perceive the direction of polarization of light. This helps them orient themselves in space with the help of sky blue. Some snakes, in turn, can perceive the IR rays, which are also invisible to us, with their pit organs.
Chemistry
In organic dyes, delocalized π-electrons can be raised to a higher level by frequencies in the visible range. This causes certain wavelengths to be absorbed, depending on the molecule.
In the case of inorganic dyes, electrons can also be excited from the d-orbitals of an atom into energetically higher d-orbitals (see ligand field theory). Furthermore, electrons can change their position between central ion and ligand within a complex (see also charge-transfer complexes and complex chemistry).
Sizes and units
- The speed of light (c) is independent of the motion of the source and decreases in media compared to the vacuum speed of light. It is 299,792,458 meters per second in a vacuum, where it is also independent of the observer's motion. The light year (Lj, ly) is the distance light travels in one year. It is used in astronomy as a unit of length.
- The colour of light is determined by the spectral composition of the light. The wavelength is inversely proportional to the energy of the light quanta.
- The polarization of light describes the orientation of the electric and magnetic field vectors of light in space. Light reflected flat on dielectric surfaces and the light of the blue sky is partially linearly polarized, while the light of incandescent lamps and the sun has no preferred direction of polarization. Linearly and circularly polarized light play a major role in optics and laser technology.
- Luminous flux (lumens) indicates how much light a light source emits in all directions.
- Luminous flux (lumen second) is the luminous flux integrated over time.
- Luminous intensity (candela) is the luminous flux per solid angle. The luminous flux can be increased by bundling.
- Luminance (candela/m²) is the luminous intensity per area of a light emitter (e.g. filament, arc, light-emitting diode).
- Illuminance (lux) describes how much light falls on a surface. It is measured with a luxmeter.
- The colour temperature (Kelvin) is the light colour of a light source equal to the temperature of a black body radiator of the same temperature in order to classify it with regard to its colour impression. The higher the value, the cooler or bluer the light.
The radiation pressure (Newton second) is the physical force effect of the light on particles or objects and plays due to its small amount hardly a role.
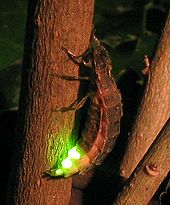

Large firefly (Lampyris noctiluca), female at midsummer lure-lighting
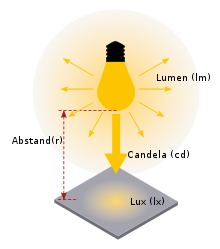

Terms of light measurement
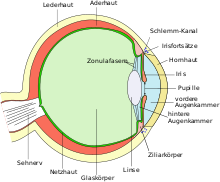

Schematic longitudinal section through the human eye
.svg.png)

Sensitivity distribution of human photoreceptors in rods (black dashed) and the three cone types (S, M and L).
Linearly polarized electromagnetic wave in vacuum. The monochromatic wave with wavelength λ propagates in the x-direction, the electric field strength
(in blue) and the magnetic flux density
(in red) are at right angles to each other and to the direction of propagation.
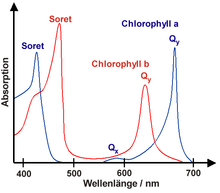

Absorption spectrum of the green leaf pigment chlorophyll a and b, with which plants can absorb and subsequently utilize light; see also Soret band


Continuous spectrum


Line spectrum (here: emission spectrum of hydrogen)
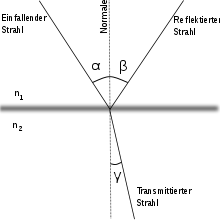

Reflection and refraction at the boundary layer of two transparent media of different optical density
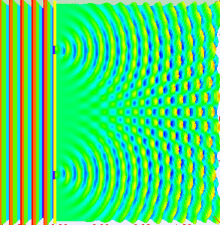

Diffraction of a plane wave at a double-slit: From each of the two slits emanates an elementary wave, which both interfere to the typical diffraction pattern of a double-slit.
Questions and Answers
Q: What is light?
A: Light is a form of electromagnetic radiation that has a wavelength which can be seen by the human eye. It also shows properties of both waves and particles, and is a form of energy made up of tiny packets called photons.
Q: What is the study of light known as?
A: The study of light is known as optics.
Q: How does light interact with opaque objects?
A: When light hits an opaque object, it makes a shadow.
Q: How does light interact with transparent objects?
A: When light hits a transparent object, it passes through it almost completely without making a shadow.
Q: What are the colours in a rainbow from outer edges to inner edges?
A: The colours in a rainbow from outer edges to inner edges are red, orange, yellow, green, blue, indigo and violet.
Q: What are wavelengths below the frequency of red called?
A: Wavelengths below the frequency of red are called infrared.
Q: What is the main source of light on Earth?
A: The main source of light on Earth is the Sun.