DNA
DNA is a redirect to this article. For other meanings, see DNA (disambiguation).
Deoxyribonucleic acid ( ; abbreviated DNA), usually abbreviated as DNA (abbreviation for deoxyribonucleic acid), is a nucleic acid composed of different deoxyribonucleotides. It carries the genetic information in all living organisms and in DNA viruses. The long-chain polynucleotide contains special sequences of its nucleotides in sections of genes. These DNA segments serve as matrices for the construction of corresponding ribonucleic acids (RNA) when genetic information is transcribed from DNA into RNA (see transcription). The RNA strands built up on the DNA template in this process fulfill different tasks; they are involved in the biosynthesis of proteins as rRNA (ribosomal RNA), as tRNA (transfer RNA) and as mRNA (messenger RNA) (see protein biosynthesis). In the case of messenger RNA (mRNA), the sequence of nucleic bases represents the building instructions for a protein.
The basic building blocks of DNA strands are four different nucleotides, each consisting of a phosphate residue, the sugar deoxyribose, and one of four nucleic bases (adenine, thymine, guanine, and cytosine; often abbreviated A, T, G, and C). The sequence of bases (base sequence) in specific DNA strand segments contains information. Transcribed into the single strand of an mRNA, its base sequence specifies the sequence of amino acids (amino acid sequence) in the protein to be formed during protein biosynthesis. In this process, three successive bases - each a base triplet as a codon - are each assigned a specific amino acid and this is linked to the previous one, so that a polypeptide is formed. In this way, areas of the base sequence are translated into an amino acid sequence on a ribosome with the aid of tRNA in accordance with the genetic code (see Translation).
The genome of a cell is usually present as a DNA double strand, in which the two base-pairing complementary strands spatially form the shape of a double helix (see figure). During replication, they are unwound and complemented separately by base pairing, so that two (almost) identical double-stranded DNA molecules are subsequently present. Errors in the replication process are a source of mutations, which are present in the resulting cells after nuclear division and cell division as a change in genetic information and can be passed on.
In the cells of eukaryotes, which include plants, animals and fungi, most of the DNA is organized in the cell nucleus (Latin nucleus, hence nuclear DNA or nDNA) as chromosomes. A small part is located in the mitochondria and is accordingly called mitochondrial DNA (mtDNA). Plants and algae also have DNA in photosynthetic organelles, the chloroplasts or plastids (cpDNA). In bacteria and archaea - the prokaryotes that do not have a nucleus - DNA is usually present in circular form in the cytoplasm (see bacterial chromosome). Some viruses store their genetic information in RNA instead of DNA (see RNA virus).
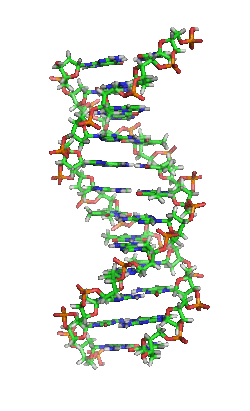

DNA helix in B conformation (structural model): The nucleic bases containing nitrogen (blue) lie horizontally between two backbone strands, which are very rich in oxygen (red). Carbon is shown in green.
Designation
The term deoxyribonucleic acid is a word composed of several components: des (from des-), oxy (from the first two syllables of oxygenium for oxygen), ribo (from the first two syllables of ribose) - thus deoxyribo (for deoxyribose) - and nucleic acid (from nucleic and acid). In German usage, deoxyribonucleic acid is now predominantly referred to as DNA using the English abbreviation for deoxyribonucleic acid, while the abbreviation DNS is considered "obsolete" according to the Duden dictionary.
Structure and organization
Building blocks
Deoxyribonucleic acid is a long chain molecule (polymer) of many building blocks called deoxyribonucleotides or nucleotides for short. Each nucleotide has three components: Phosphoric acid or phosphate, the sugar deoxyribose, and a heterocyclic nucleobase or base for short. The deoxyribose and phosphoric acid subunits are the same for each nucleotide. They form the backbone of the molecule. Units of base and sugar (without phosphate) are called nucleosides.
The phosphate residues are hydrophilic due to their negative charge, they give DNA in aqueous solution an overall negative charge. Since this negatively charged DNA dissolved in water cannot release any further protons, strictly speaking it is not (any longer) an acid. The term deoxyribonucleic acid refers to an uncharged state in which protons are attached to the phosphate residues.
The base can be a purine, namely adenine (A) or guanine (G), or a pyrimidine, namely thymine (T) or cytosine (C). Since the four different nucleotides differ only by their base, the abbreviations A, G, T and C are also used for the corresponding nucleotides.
The five carbon atoms of a deoxyribose are numbered from 1' (read one dash) to 5'. The base is attached to the 1' end of this sugar. The phosphate residue is attached to the 5' end. Strictly speaking, the deoxyribose is the 2-deoxyribose; the name comes from the fact that, compared to a ribose molecule, an alcoholic hydroxy group (OH group) is missing at the 2'-position (i.e. replaced by a hydrogen atom).
An OH group is present at the 3' position, which links the deoxyribose to the 5' carbon atom of the sugar of the next nucleotide via a so-called phosphodiester bond (see figure). As a result, each so-called single strand has two different ends: a 5' and a 3' end. DNA polymerases, which carry out the synthesis of DNA strands in the living world, can only add new nucleotides to the OH group at the 3' end, but not at the 5' end. Thus, the single strand always grows from 5' to 3' (see also DNA replication below). In this process, a nucleoside triphosphate (with three phosphate residues) is delivered as a new building block, from which two phosphates are cleaved in the form of pyrophosphate. The remaining phosphate residue of each newly added nucleotide is linked to the OH group at the 3' end of the last nucleotide present in the strand with water splitting. The sequence of bases in the strand encodes the genetic information.
The double helix
DNA normally occurs as a helical double helix in a conformation called B-DNA. Two of the single strands described above are attached to each other in opposite directions: At each end of the double helix, one of the two single strands has its 3' end, the other its 5' end. As a result of the juxtaposition, two particular bases are always opposite each other in the middle of the double helix, they are "paired". The double helix is stabilized mainly by stacking interactions between successive bases of the same strand (and not, as often claimed, by hydrogen bonds between strands).
Adenine and thymine always pair up, forming two hydrogen bonds, or cytosine with guanine, which are connected by three hydrogen bonds. Bridging occurs between the molecular positions 1═1 and 6═6, and in the case of guanine-cytosine pairings additionally between 2═2. Since the same bases always pair, the sequence of the bases in one strand can be used to deduce that of the other strand; the sequences are complementary (see also: base pair). Here, the hydrogen bonds are almost exclusively responsible for the specificity of the pairing, but not for the stability of the double helix.
Since a purine is always combined with a pyrimidine, the distance between the strands is the same everywhere, resulting in a regular structure. The whole helix has a diameter of about 2 nm and winds further by 0.34 nm with each sugar molecule.
The planes of the sugar molecules are at an angle of 36° to each other, and complete rotation is consequently achieved after 10 bases (360°) and 3.4 nm. DNA molecules can become very large. For example, the largest human chromosome contains 247 million base pairs.
When the two single strands wind around each other, lateral gaps remain, so that here the bases lie directly on the surface. There are two of these furrows that wind around the double helix (see figures and animation at the beginning of the article). The "large furrow" is 2.2 nm wide, the "small furrow" only 1.2 nm.
Accordingly, the bases in the major groove are more accessible. Proteins that bind to DNA in a sequence-specific manner, such as transcription factors, therefore usually bind to the major furrow.
Some DNA dyes, such as DAPI, also attach to a furrow.
The cumulative binding energy between the two single strands holds them together. Covalent bonds are not present here, so the DNA double helix consists of two molecules rather than one. This allows the two strands to be temporarily separated in biological processes.
In addition to the B-DNA just described, there is also A-DNA and a left-handed, so-called Z-DNA, which was studied for the first time in 1979 by Alexander Rich and his colleagues at MIT. This occurs particularly in G-C-rich segments. It was not until 2005 that a crystal structure was reported showing Z-DNA directly in a junction with B-DNA, thus providing evidence for a biological activity of Z-DNA. The following table and the adjacent figure show the differences of the three forms in direct comparison.
Structural information of the three DNA forms that could be biologically relevant | |||
Structure feature | A-DNA | B-DNA | Z-DNA |
Structure from | Monomers | Monomers | Dimer |
Direction of rotation of the helix | right | right | left |
Diameter (approx.) | 2.6 nm | 2.37 nm | 1.8 nm |
Helical turn per base pair (twist) | 32,7° | 34,3° | 30° |
Base pairs per helical turn | 11 | 10 | 12 |
Increase per base | 0.29 nm | 0.34 nm | 0.37 nm |
Rise per turn (gear height) | 3.4 nm | 3.4 nm | 4.4 nm |
Angle of inclination of the base pairs to the axis | 20° | 6° | 7° |
Large furrow | narrow and deep | wide and deepDepth | flat |
Small furrow | wide and flat | Narrow and deepDepth | narrow and deep |
Pyrimidine bases (cytosine/thymine/uracil) |
|
| C2'-endo |
Purine bases (adenine/guanine) |
|
| C3'-endo |
The stacks of base pairs (base stackings) do not lie exactly parallel to each other like books, but form wedges that tilt the helix in one direction or the other. The largest wedge is formed by adenosines paired with thymidines of the other strand. Consequently, a series of AT pairs forms an arc in the helix. When such series follow each other at short intervals, the DNA molecule adopts a bent or a curved structure, which is stable. This is also called sequence-induced diffraction, because the diffraction can also be caused by proteins (the so-called protein-induced diffraction). Sequence-induced diffraction is often found at important sites in the genome.
Chromatin and chromosomes
→ Main article: Chromatin and chromosome
DNA in the eukaryotic cell is organized in the form of chromatin strands called chromosomes, which are located in the nucleus. A single chromosome contains a long, continuous DNA double strand (in a chromatid) from anaphase to the beginning of S phase. At the end of S phase, the chromosome consists of two identical DNA strands (in two chromatids).
Since such a DNA thread can be several centimeters long, but a cell nucleus has a diameter of only a few micrometers, the DNA must be additionally compressed or "packed". In eukaryotes, this is done with so-called chromatin proteins, of which the basic histones deserve special mention. They form the nucleosomes around which the DNA is wrapped at the lowest packaging level. During nuclear division (mitosis), each chromosome is condensed to its maximum compact form. This allows them to be identified particularly well in metaphase with the light microscope.
Bacterial and viral DNA
In prokaryotic cells, the majority of double-stranded DNA in the cases documented so far is not present as linear strands, each with a beginning and an end, but as circular molecules - each molecule (i.e. each DNA strand) closes into a circle with its 3' and its 5' end. These two circular, closed DNA molecules are called bacterial chromosomes or plasmids, depending on the length of the sequence. In bacteria, they are also not located in a nucleus, but are freely present in the plasma. The prokaryote DNA is wound up with the help of enzymes (for example, topoisomerases and gyrases) into simple "supercoils" that resemble a curled telephone cord. By twisting the helices around themselves, the space required for the genetic information is reduced. In bacteria, topoisomerases ensure that the twisted double strand is untwisted at a desired position by constantly cutting and rejoining the DNA (a prerequisite for transcription and replication). Depending on their type, viruses contain either DNA or RNA as genetic information. In both DNA and RNA viruses, the nucleic acid is protected by a protein envelope.
Chemical and physical properties of the DNA double helix
DNA is a negatively charged molecule at neutral pH, with the negative charges sitting on the phosphates in the backbone of the strands. Although two of the three acidic OH groups of the phosphates are esterified with the respective adjacent deoxyriboses, the third is still present and releases a proton at neutral pH, causing the negative charge. This property is exploited in agarose gel electrophoresis to separate different DNA strands according to their length. Some physical properties such as the free energy and the melting point of the DNA are directly related to the GC content, i.e. they are sequence-dependent.
Stacking interactions
Two main factors are responsible for the stability of the double helix: base pairing between complementary bases and stacking interactions between consecutive bases.
Contrary to initial assumptions, the energy gain from hydrogen bonds is negligible, since the bases can form similarly good hydrogen bonds with the surrounding water. The hydrogen bonds of a GC base pair contribute only minimally to the stability of the double helix, while those of an AT base pair even have a destabilizing effect. Stacking interactions, on the other hand, act only in the double helix between consecutive base pairs: A dipole-induced dipole interaction occurs between the aromatic ring systems of the heterocyclic bases, which is energetically favorable. Thus, the formation of the first base pair is quite unfavorable due to the low energy gain and loss, but the elongation (lengthening) of the helix is energetically favorable because the base pair stacking proceeds under energy gain.
However, the stacking interactions are sequence-dependent and energetically most favorable for stacked GC-GC, less favorable for stacked AT-AT. The differences in stacking interactions mainly explain why GC-rich DNA segments are thermodynamically more stable than AT-rich ones, while hydrogen bonding plays a minor role.
Melting point
The melting point of DNA is the temperature at which the binding forces between the two single strands are overcome and they separate from each other. This is also referred to as denaturation.
As long as DNA denatures in a cooperative transition (which occurs in a narrow temperature range), the melting point refers to the temperature at which half of the double strands have denatured into single strands. The correct terms "midpoint of transition temperature" or midpoint temperature Tm are derived from this definition.
The melting point depends on the respective base sequence in the helix. It increases when there are more GC base pairs in it, since these are entropically more favorable than AT base pairs. This is not so much due to the different number of hydrogen bonds formed by the two pairs, but much more to the different stacking interactions. The stacking energy of two base pairs is much smaller when one of the two pairs is an AT base pair. GC stacks, on the other hand, are more energetically favorable and stabilize the double helix more strongly. The ratio of GC base pairs to the total number of all base pairs is given by the GC content.
Since single-stranded DNA absorbs UV light about 40 percent more strongly than double-stranded DNA, the transition temperature can be easily determined in a photometer.
When the temperature of the solution falls back below Tm, the individual strands can reattach to each other. This process is called renaturation or hybridization. The interplay of de- and renaturation is exploited in many biotechnological processes, for example in the polymerase chain reaction (PCR), Southern blots and in situ hybridization.
Cruciform DNA on palindromes
A palindrome is a sequence of nucleotides in which the two complementary strands can be read in the same way from the right as from the left.
Under natural conditions (with high torsional stress of the DNA) or artificially in the test tube, this linear helix can form as a cruciform by creating two branches that protrude from the linear double strand. The branches each represent a helix in their own right, but at least three nucleotides remain unpaired at the end of a branch. During the transition from the cross form to the linear helix, base pairing is maintained because of the bending ability of the phosphodiester-sugar backbone.
The spontaneous assembly of complementary bases into so-called stem-loop structures is also frequently observed in single-stranded DNA or RNA.
Non-standard bases
Occasionally, deviations from the above four canonical bases (standard bases) adenine (A), guanine (G), thymine (T), and cytosine (C) are observed in viruses and cellular organisms; further deviations can be artificially generated.
Natural non-standard bases
- Uracil (U) is not normally found in DNA; it occurs only as a degradation product of cytosine. However, in several bacteriophages (bacterial viruses), thymine is replaced by uracil:
- Bacillus subtilis bacteriophage PBS1 (ICTV: species Bacillus virus PBS1) and "PBS2" (proposed species 'Bacillus phage PBS2' aka "Bacteriophage PBS2") - both species are myophages, i.e. phages from the family Myoviridae (without assigned genus).
- Bacillus virus PBS1 (ICTV: species Yersinia virus R1RT in genus Tg1virus, family Myoviridae).
- Staphylococcus phage S6 (aka Staphylococcus aureus bacteriophage 15, also from the family Myoviridae)
Uracil is also found in the
DNA of eukaryotes such as Plasmodium falciparum (Apicomplexa). It is present there in relatively small amounts (7-10 uracil units per million bases). - 5-Hydroxymethyldesoxyuridine (hm5dU) replaces thymidine in the genome of various Bacillus phages of the species Bacillus virus SPO1, genus Spo1virus (formerly spounalikevirus or SPO1-like viruses), also family Myoviridae. These are the phages SPO1, SP8, SP82, "Phi-E" alias "ϕe" and "2C").
- 5-Dihydroxypentauracil (DHPU, with nucleotide 5-dihydroxypentyl-dUMP, DHPdUMP) was described as a replacement for thymidine in "Bacillus phage SP15" (also "SP-15", family Myoviridae).
- Beta-d-glucopyranosyloxymethyluracil (base J), also a modified form of uracil, was found in several organisms: The flagellates Diplonema and Euglena (both Excavata: Euglenozoa) and all genera of kinetoplastids. The biosynthesis of J occurs in two steps: In the first step, a specific thymidine in DNA is converted to hydroxymethyldesoxyuridine (HOMedU), and in the second step, HOMedU is glycosylated to J. There are some proteins that specifically bind to this base. These proteins appear to be distant relatives of the Tet1 oncogene, which is involved in the pathogenesis of acute myeloid leukemia. J appears to act as a termination signal for RNA polymerase II.
- 2,6-Diaminopurine (a.k.a. 2-aminoadenine, base D or X, DAP): In 1976, it was found that the "Cyanophage S-2L" (a.k.a. "Cyanobacteria phage S-2L", genus "Cyanostylovirus", family Siphoviridae, possibly own family "Cyanostyloviridae" or "Styloviridae"), whose hosts are species of the genus Synechocystis, replaced all adenosine bases in its genome with 2,6-diaminopurine. Three additional studies followed in 2021, and a summary can be found on sciencealert (May 2021). A similar situation applies to "Acinetobacter phage SH-Ab 15497", also Siphoviridae, and other representatives of this family as well as Podoviridae.
- As found in 2016, 2'-deoxyarchaeosin (dG+) is present in the genome of several bacteria and in Escherichia phage 9g (ICTV: Escherichia virus 9g, genus Nonagvirus, family Siphoviridae).
- 6-methylisoxanthopterin
- 5-Hydroxyuracil
Natural modified bases (methylations etc.)
Modified bases also occur in natural DNA. In particular, methylations of the canonical bases are studied in the context of epigenetics:
- First, in 1925, 5-methylcytosine (m5C) was found in the genome of Mycobacterium tuberculosis. In the genome of Xanthomonas oryzae bacteriophage Xp12 (Xanthomonas phage XP-12, family Siphoviridae) and halovirus ΦH (Halobacterium virus phiH, genus Myohalovirus, Myoviridae), the entire cystosine contingent is replaced by 5-methylcytosine.
- A complete replacement of cytosine by 5-glycosylhydroxymethylcytosine (syn. glycosyl-5-hydroxymethylcytosine) in phages T2, T4, and T6 of the species Escherichia virus T4 (genus Tquattrovirus, subfamily Tevenvirinae of the family Myoviridae) was observed in 1953.
- As discovered in 1955, N6-methyladenine (6mA, m6A) is present in the DNA of coliform bacteria.
- N6-carbamoylmethyladenine was described in 1975 in bacteriophage Mu (ICTV: species Escherichia virus Mu, formerly Enterobacteria phage Mu; genus Muvirus, obsolete Mulikevirus in the family Myoviridae) and Lambda-Mu.
- 7-Methylguanine (m7G) was described in 1976 in phage DDVI ('Enterobacteria phage DdVI' aka 'DdV1', genus T4virus) of Shigella disenteriae.
- N4-methylcytosine (m4C) in DNA was described in 1983 (in Bacillus centrosporus).
- In 1985, 5-hydroxycytosine was found in the genome of Rhizobium phage RL38JI.
- α-Putrescinylthymine (alpha-putrescinylthymine, putT) and α-glutamylthymidine (alpha-glutamylthymidine) are present in the genome of both Delftia phage ΦW-14 (Phi W-14, species 'Dellftia virus PhiW14', genus Ionavirus, family Myovrirdae) and Bacillus phage SP10 (also family Myoviridae).
- 5-Dihydroxypentyluracil was found in Bacillus phage SP15 (also SP-15, family Myoviridae).
The function of these non-canonical bases in DNA is not known. They act, at least in part, as a molecular immune system and help protect the bacteria from infection by viruses.
However, non-standard and modified bases in microbes are not the whole story:
- Four modifications of cytosine residues in human DNA have also been reported. These modifications consist of the addition of the following groups:
- Methyl (-CH3)
- Hydroxymethyl (-CH2OH)
- Formyl (-CHO)
- Carboxyl (-COOH)
It is assumed that these modifications have regulatory functions, keyword epigenetics. - Uracil is found in the centromere regions of at least two human chromosomes (6 and 11).
Synthetic bases
In the laboratory, DNA (and also RNA) was provided with additional artificial bases. The aim is usually to create unnatural base pairs (UBP):
- In 2004, DNA was generated that contained an expanded alphabet with six nucleobases (A, T, G, C, dP, and dZ) instead of the four standard nucleobases (A, T, G, and C). Here, for these two new bases, dP represents 2-amino-8-(1′-β-D-2′-deoxyribofuranosyl)-imidazo[1,2-a]-1,3,5-triazin-4(8H)-one and dZ represents 6-amino-5-nitro-3-(1′-β-D-2′-deoxyribofuranosyl)-2(1H)-pyridone.
- In 2006, DNA with bases extended by a benzene group or a naphthyl group (called either xDNA or xxDNA or yDNA or yyDNA, depending on the position of the extension groups) was studied for the first time.
- Yorke Zhang et al. reported at the turn of 2016/2017 semisynthetic organisms with DNA extended to include bases X (a.k.a. NaM) and Y' (a.k.a. TPT3) or the (deoxyribo) nucleotides dX (dNaM) and dY' (dTPT3) that pair with each other. This was preceded by experiments with pairings based on bases X and Y (aka 5SICS), i.e., nucleotides dX and dY (aka d5SICS). Other bases that can pair with 5SICS are FEMO and MMO2.
- In early 2019, DNA and RNA were reported to have eight bases each (four natural and four synthetic), all of which map to each other in pairs (Hachimoji DNA).
Enantiomers
DNA occurs in living organisms as D-DNA; however, L-DNA can be synthesized as an enantiomer (mirror) (the same applies analogously to RNA). L-DNA is degraded more slowly by enzymes than the natural form, which makes it interesting for pharmaceutical research.
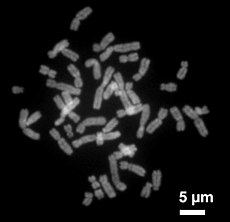

Human chromosomes in late metaphase of mitotic nuclear division: each chromosome shows two chromatids, which are separated and split for two nuclei in anaphase.
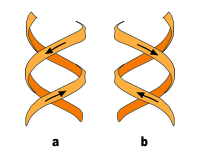

a) right-handed double helixb ) left-handed double helix
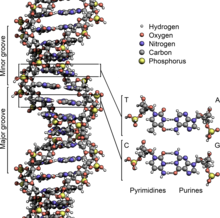

DNA Structure Key Labelled NoBB
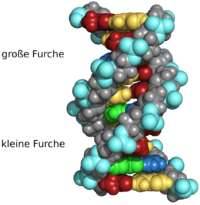

Section of a DNA molecule: The dome model used here better represents the occupation of the volume of space and avoids the impression that there is still a lot of space between the atoms. However, bonds between the atoms are represented worse.
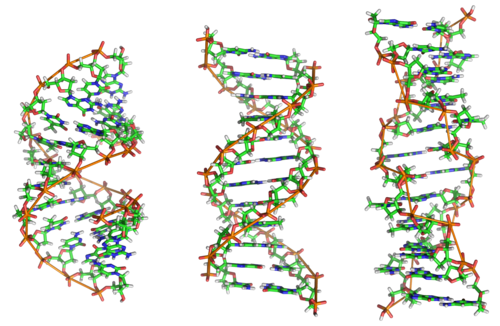

A-, B- and Z-DNA: structural models with 12 base pairs each (f. l. t. r.)
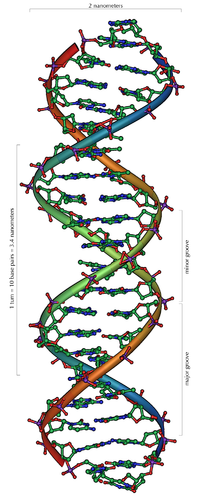

Section of 20 base pairs from the DNA double helix (B-form; structural model)
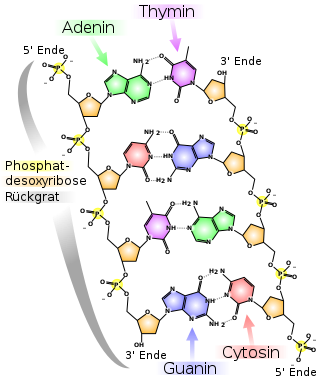

Structural formula of a DNA excerpt
Genetic information content and transcription
→ Main article: Gene, Genetic Code and Transcription (Biology)
DNA molecules play an important role as information carriers and "docking sites" for enzymes that are responsible for transcription. Furthermore, the information of certain DNA segments, as present in operative units such as the operon, is important for regulatory processes within the cell.
Certain sections of DNA, known as genes, encode genetic information that influences the structure and organization of the organism. Genes contain "blueprints" for proteins or molecules that are involved in protein synthesis or the regulation of a cell's metabolism. The sequence of the bases determines the genetic information. This base sequence can be determined by sequencing, for example using the Sanger method.
The base sequence of a gene segment of DNA is first transcribed into the complementary base sequence of a so-called ribonucleic acid molecule (abbreviated RNA). Unlike DNA, RNA contains the sugar ribose instead of deoxyribose and the base uracil instead of thymine, but the information content is the same. For protein synthesis, so-called mRNAs are used, single-stranded RNA molecules that are transported out of the cell nucleus into the cytoplasm, where protein synthesis takes place (see protein biosynthesis).
According to the so-called "one-gene-one-protein hypothesis", the sequence of one protein molecule is read from a coding section on the DNA. However, there are regions of DNA that each encode several proteins by using different reading frames during transcription. In addition, different isoforms of a protein can be produced by alternative splicing (subsequent cutting of the mRNA).
In addition to coding DNA (the genes), there is non-codingDNA, which in humans, for example, makes up more than 90 percent of a cell's total DNA.
The storage capacity of DNA has not yet been technically replicated. With the information density in a teaspoon of dried DNA, the current world population could be replicated about 350 times.
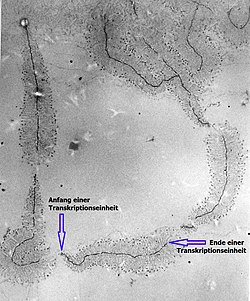

Ribosomal DNA in transcription: the length of newly synthesized rRNA molecules increases from the beginning to the end of a transcription unit (electron micrograph, magnification 40,000 times).
DNA Replication
→ Main article: Replication
DNA can duplicate itself (replicate) according to the so-called semiconservativeprinciple with the help of enzymes. The double-stranded helix is separated by the enzyme helicase after it has been despiralized by topoisomerase. The resulting single strands serve as a template for the complementary counterstrand to be synthesized, which is attached to them.
DNA synthesis, i.e. the binding of the nucleotides to be linked, is carried out by enzymes from the group of DNA polymerases. A nucleotide to be linked must be present in the triphosphate compound - i.e. as a deoxyribonucleoside triphosphate. By splitting off two phosphate moieties, the energy required for the binding process is released.
The enzyme helicase forms a replication fork, two DNA single strands running apart. In their area, an RNA primer synthesized by the enzyme primase marks the starting point of DNA re-synthesis. To this RNA molecule, the DNA polymerase successively attaches nucleotides that are complementary to those of the DNA single strands.
The linkage of the new nucleotides to form a complementary DNA single strand can only proceed in the 5'→3' direction on the two old strands and consequently does so without interruption along the old 3'→5' strand toward the ever-opening replication fork.
The synthesis of the new strand on the old 5'→3' strand, on the other hand, cannot take place continuously toward the replication fork, but only away from it, also in the 5'→3' direction. However, the old double strand is only open a short distance at the beginning of replication, so that only a short piece of new complementary DNA can be formed on the second strand - in the "inappropriate" opposite direction.
Since a DNA polymerase only links about 1000 nucleotides at a time, it is necessary to synthesize the entire complementary strand in individual pieces. When the replication fork has opened a little further, a new RNA primer therefore attaches itself again directly to the second single strand at the fork site and initiates the next DNA polymerase.
In the synthesis of the 3'→5' strand, a new RNA primer is therefore required for each DNA synthesis unit. Primer and associated synthesis unit are referred to as Okazaki fragment. The RNA primers required for the replication start are subsequently degraded enzymatically. This creates gaps in the new DNA strand, which are filled with DNA nucleotides by special DNA polymerases.
Finally, the enzyme ligase links the new DNA segments that have not yet been joined together to form a single strand.
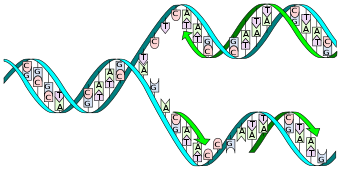

The double helix is opened by the helicase and the topoisomerase. Then the primase sets a primer and the DNA polymerase starts to copy the leading strand. A second DNA polymerase binds the subsequent strand, but cannot synthesize continuously; instead, it produces individual Okazaki fragments that are joined together by the DNA ligase.
Mutations and other DNA damage
→ Main article: Mutation, DNA damage and DNA repair.
Mutations of DNA segments - for example, exchange of bases for others or changes in the base sequence - lead to changes in the genetic material, some of which can be lethal for the affected organism.
In rare cases, however, such mutations are also advantageous; they then form the starting point for the modification of living beings in the course of evolution. By means of recombination during sexual reproduction, this change in DNA even becomes a decisive factor in evolution: the eukaryotic cell usually has several sets of chromosomes, i.e. a DNA double strand is present at least twice. Through the mutual exchange of parts of these DNA strands, the crossing-over during meiosis, new characteristics can thus arise.
DNA molecules can be damaged by various influences. Ionizing radiation, such as UV or γ-radiation, alkylation as well as oxidation can chemically change the DNA bases or lead to strand breakage. These chemical changes may affect the pairing properties of the affected bases. Many of the mutations during replication occur in this way.
Some common DNA damage includes:
- the formation of uracil from cytosine with spontaneous loss of an amino group by hydrolysis: like thymine, uracil is complementary to adenine.
- Thymine-thymine dimer damage caused by photochemical reaction of two consecutive thymine bases in the DNA strand by UV radiation, for example from sunlight. This damage is probably a major cause of skin cancer.
- the formation of 8-oxoguanine by oxidation of guanine: 8-oxoguanine is complementary to both cytosine and adenine. During replication, both bases can be incorporated opposite 8-oxoguanine.
Due to their mutagenic properties and their frequent occurrence (estimates are 104 to 106 new damages per cell per day), DNA damage must be removed from the genome in a timely manner. Cells have an efficient DNA repair system for this purpose. It removes damage using the following strategies:
- Direct damage reversion: An enzyme reverses the chemical change at the DNA base.
- Base excision repair: The faulty base, for example 8-oxoguanine, is excised from the genome. The resulting vacant site is re-synthesized using the information in the opposite strand.
- Nucleotide excision repair: A larger strand containing the damage is excised from the genome. This is re-synthesized using the information in the opposite strand.
- Homologous recombination: If both DNA strands are damaged, the genetic information from the second chromosome of the homologous chromosome pair is used for repair.
- Replication with special polymerases: DNA polymerase η, for example, can replicate error-free via TT dimer damage. People in whom polymerase η does not function or functions only to a limited extent often suffer from xeroderma pigmentosum, a hereditary disease that leads to extreme sensitivity to sunlight.
Denaturation
The base pairing of DNA is denatured during various cellular processes. In this process, the base pairing is abolished section by section by various DNA-binding proteins, e.g. during replication or transcription. The site of denaturation onset is called the denaturation bubble and is described in the Poland-Scheraga model. However, DNA sequence, stiffness, and torsion are not included. The lifetime of a denaturation bubble is between one microsecond and one millisecond.
In the laboratory, DNA can be denatured by physical and chemical methods. DNA is denatured by formamide, dimethylformamide, guanidinium salts, sodium salicylate, sulfoxide, dimethyl sulfoxide (DMSO), various alcohols, propylene glycol and urea, usually in combination with heat. Concentrated solutions of sodium hydroxide also denature DNA. Chemical methods involve lowering the melting temperature of double-stranded DNA.
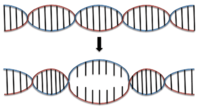

Beginning DNA denaturation
DNA purification and detection
DNA can be separated from other biomolecules by DNA purification, e.g. by DNA extraction. Qualitative detection of DNA (what DNA is present) is usually done by a polymerase chain reaction, isothermal DNA amplification, DNA sequencing, Southern blot, or in situ hybridization. Quantitative detection (how much DNA is present) is most often done by qPCR; for purified samples with only one DNA sequence, a concentration can also be measured by photometry at a wavelength of 260 nm. An absorbance of 1 of a purified DNA solution corresponds to a concentration of 50 µg/mL for double-stranded DNA, for single-stranded DNA this corresponds to 33 µg/mL and for single-stranded oligonucleotides the concentration is lower, depending on the composition of nucleic bases (see DNA extraction#Quantification). DNA can be stained by intercalating dyes such as ethidium bromide, propidium iodide or SYBR Green I as well as by furrow-binding dyes such as DAPI, Pentamidine, Lexitropsine, Netropsine, Distamycin, Hoechst 33342 or Hoechst 33258. Less specifically bound DNA dyes and staining methods include methylene blue, the carbocyanine dye Stains-All, or silver staining. Molecular combining can be used to stretch and align DNA.
"Old" DNA
aDNA ("ancient DNA") is the term used to describe the remains of genetic material molecules in dead organisms when no direct relatives of the sampled organism are still alive. Human DNA is also referred to as aDNA if the individual died at least 75 years before the sample was examined.
See also
- Xenonucleic acid, XNA, in addition:
· LNA
· Peptide Nucleic Acid (PNA)
· Morpholino
· Dideoxyribonucleoside triphosphates (ddNTPs): Artificial intermediates in Sanger DNA sequencing.
- Deoxyadenosine monoarsenate (dAMAs) see GFAJ-1 §Discussion about the incorporation of arsenic into biomolecules (questionable incorporation into DNA in Halomonas species GFAJ-1, see also Halomonas titanicae).
Questions and Answers
Q: What is DNA?
A: DNA stands for deoxyribonucleic acid and is the molecule that contains the genetic code of organisms, including animals, plants, protists, archaea and bacteria. It is made up of two polynucleotide chains in a double helix.
Q: How does DNA tell cells what proteins to make?
A: Mostly, the proteins that are made are enzymes which are determined by the instructions contained within the DNA.
Q: How do children inherit traits from their parents?
A: Children share traits with their parents because they inherit part of their parent's DNA which determines things like skin, hair and eye color. The combination of both parent's DNA forms a unique set of instructions for each child.
Q: What is non-coding DNA?
A: Non-coding DNA sequences are parts of an organism's genome that do not code for protein sequences. Some non-coding DNAs can be transcribed into non-coding RNA molecules such as transfer RNA or ribosomal RNA while other sequences may not be transcribed at all or give rise to RNAs with unknown functions. The amount of non-coding DNAs varies among species.
Q: Where do eukaryotic organisms store most of their DNA?
A: Eukaryotic organisms like animals, plants, fungi and protists store most of their DNAs inside the cell nucleus while prokaryotes like bacteria and archaea store theirs only in the cytoplasm in circular chromosomes.
Q: How does chromatin help organize DNA inside eukaryotic chromosomes?
A: Chromatin proteins such as histones help compact and organize DNAs inside eukaryotic chromosomes so it can be easily accessed when needed.